
The global point-of-care diagnostic market is projected to reach $75 billion by 2027, with a Compound Annual Growth Rate (CAGR) exceeding 10%. This is driven by the urgent need for rapid diagnostic tests for detecting infectious diseases and other factors, such as an aging population and the rise in chronic diseases.
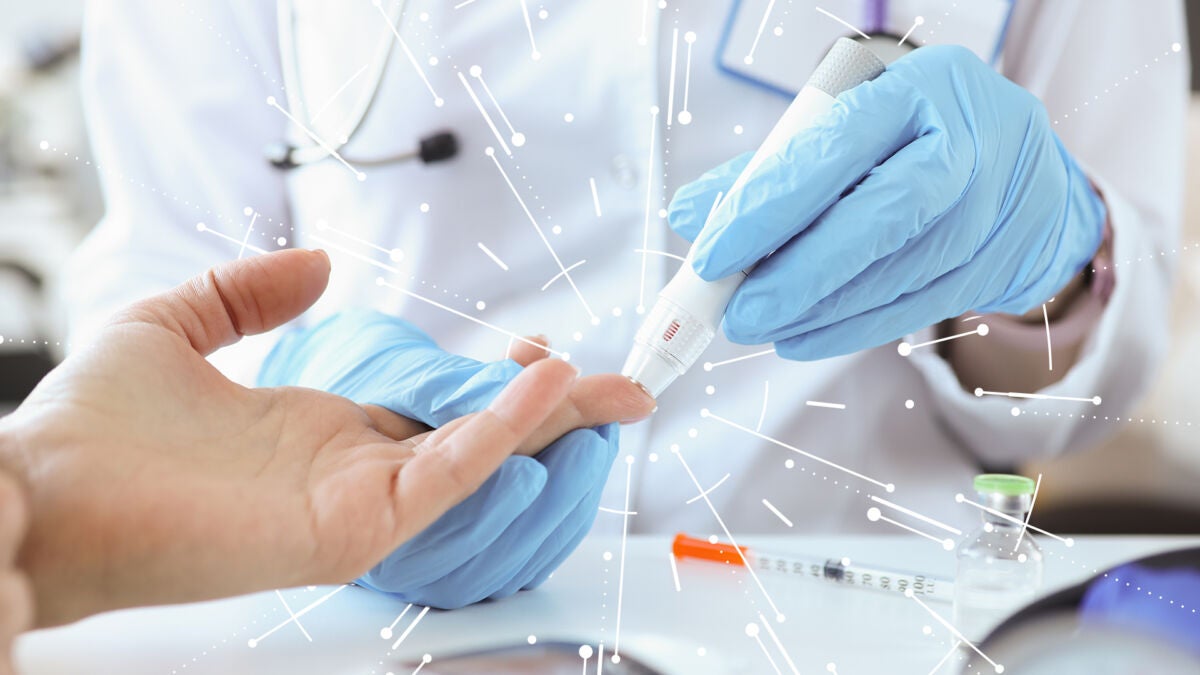
Point-of-care diagnostics are tests that are performed near or close to the patient’s location, enabling rapid diagnoses and treatment decisions. Examples include blood glucose monitoring, home pregnancy tests, and tests for hemoglobin, fecal occult blood, prothrombin time, cardiac markers, urine markers, and infectious disease testing such as COVID-19.
Point of care diagnostic system design
Designing point-of-care diagnostic systems presents several critical considerations for engineering teams. These include ensuring measurement accuracy and reliability, user-friendly operation, and scalable production with validated manufacturing processes. In typical point-of-care diagnostic solutions, a durable system reader manages the entire measurement cycle, while within a disposable cassette, the combination of the sample with specific reagents amplifies the analyte and prepares it for further detection.
These are the typical test steps
Collect
- Sample is taken via a dip stick, swab, capillary collection tube
- Transferred and diluted into a first reservoir
Measure
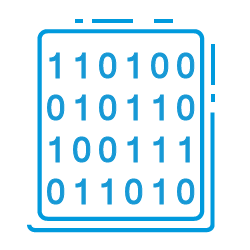
- Sample is taken via a dip stick, swab, capillary collection tube
- Sample is taken via a dip stick, swab, capillary collection tube
Separate
- Filtering of particles
- Extraction of RNA, DNA to be prepared for amplification
Display
- Machine status
- Display of results
Amplify
- RT-PCR, RT-LAMP and other methods
- Replication of the target analyte to increase its concentration
- Tagging with a marker to enable read out
Transfer
- Cloud data transfer
- RF communication
- Data Integrity and security protocols
Transduce
- Excitation of the marker
- Generation of an optical signal proportional to the concentration of the analyte
Next, we will dive into each step—collection, separation, amplification, transduction, measurement, display, and transfer—to go over key functions, challenges, and solutions.
Collection
Obtaining a sample from the patient and depositing it into a reservoir for subsequent processing requires a reliable way to maintain both sterility and ease of use. It is crucial to ensure that the tools used for sample collection are sterile to prevent contamination and ensure accurate results. Additionally, designing a user-friendly experience that is intuitive, simple, and reliable is essential to enhance the usability of the diagnostic system.
To address the challenge of sterile sample collection, engineering teams can focus on integrating components into the disposable cartridge. By reducing the number of manual operations required by the end user, the risk of contamination can be minimized. This integration also contributes to the reduction of assembly and packaging costs.
To maintain consistency in sample size and solvent volume, a secondary test can be conducted. This test provides an independent signal proportional to the concentration of the collected material, such as DNA. By compensating for variations in sample size and solvent volume, the accuracy of the measurement can be enhanced.
Separation
After collection, the sample is transferred into a disposable cartridge, which functions as a miniature diagnostic lab. In this step, the fluid architecture must ensure efficient movement of the liquid while preventing disruptions caused by impurities or the presence of particulates brought into the solution by the swab. Additionally, the thermal interface between the cartridge and the reader needs to be carefully designed to achieve controlled temperature conditions for the lysis process.
Different fluidic architectures can be employed to ensure efficient liquid movement. Gravity-based systems utilize valves to control the flow, while disk-shaped cartridges employ centrifugal force. Some systems incorporate pumps within the reader or build micropumps into the cartridge to create pressure differentials. Advanced solutions
leverage capillary forces and microfluidic channels for precise liquid navigation. Filtering mechanisms can also be implemented to remove impurities before the liquid progresses through the system.
For the thermal interface, precise tolerances and a lack of air gaps between the heating blocks in the reader and the well in the cartridge are crucial. The use of a soft, thermally conductive rubber mat can improve the thermal interface. Temperature sensors placed in the reader help achieve controlled temperature conditions during the lysis process.
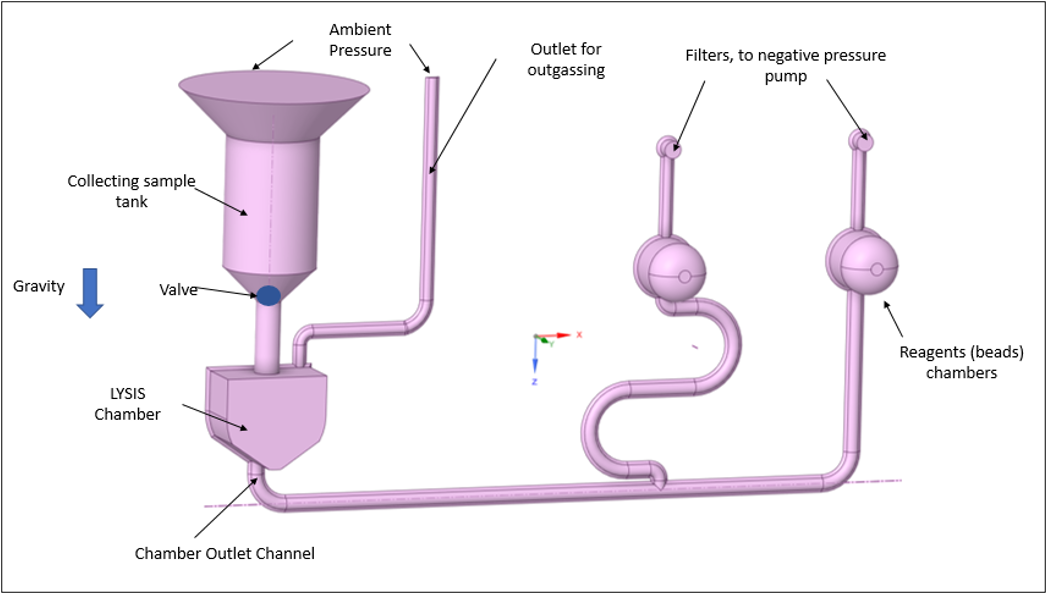
Figure 1 – Example of a fluidic structure and main elements in a cartridge
The subsequent steps after lysis require the transfer of the solution into a new well and the design of fluidic channels to ensure precise liquid movement without the entrapment of air bubbles. In cases of multi-well architectures, equal distribution of the liquid among the branches and simultaneous well filling need to be ensured.
To achieve precise liquid movement, micropumps in the reader can be utilized to create pressure differentials. Electrodes placed at different gate points along the path can inform the electronics about the liquid’s position in the cartridge.
Designing fluidic features, including wells, valves, and channels, requires careful engineering and optimization. Recent advancements in 3D microfluidic simulations can significantly reduce the time needed to optimize the cartridge architecture, allowing for more efficient design iterations.
Addressing the challenges in fluidic architecture and thermal interface design involves employing various fluidic architectures, implementing filtering mechanisms, optimizing the thermal interface, utilizing micropumps and electrodes, and employing blocking membranes. These solutions contribute to efficient sample processing and precise liquid movement in point-of-care diagnostic systems.
Amplification
In this step, the point-of-care diagnostic device must amplify the low concentration of the analyte using PCR-based technology or other molecular means. Reagents used in the amplification process must undergo checks for sensitivity, specificity, and thermal effects. Reagent degradation and moisture sensitivity are key manufacturing issues, especially when using pre-lyophilized reagents in the form of beads. The cassette assembly must be done in a controlled low humidity environment and sealed with moisture barriers and desiccants to prevent reagent degradation.
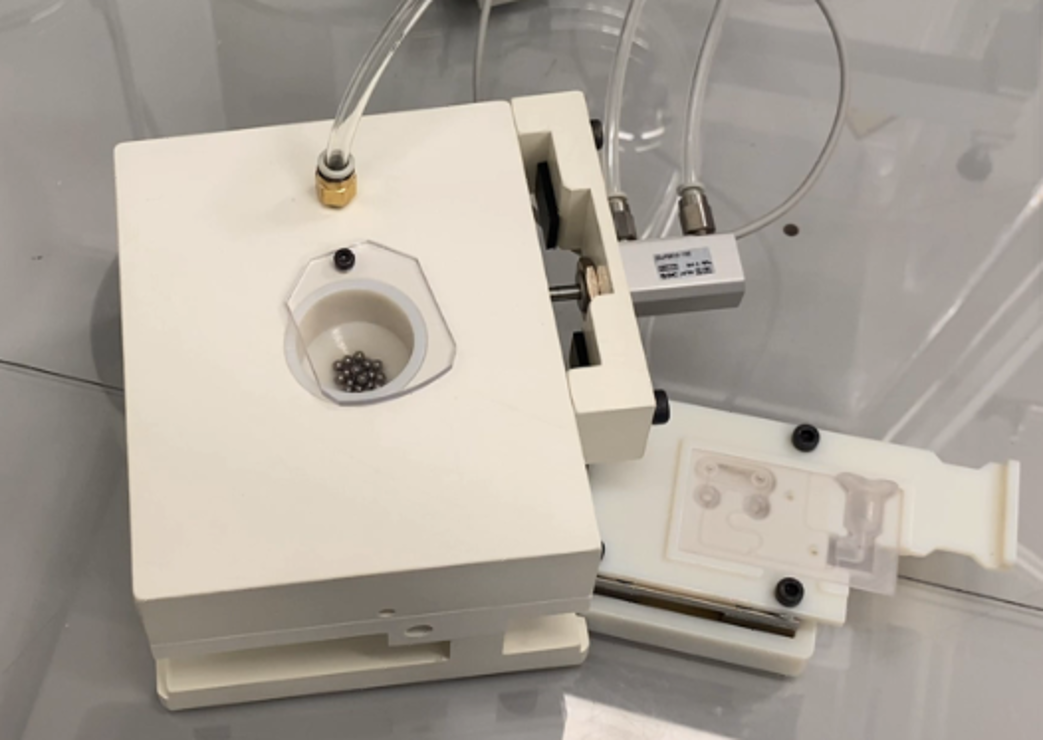
The selection of cartridge material and its wettability is crucial to control the flow speed from well to well. The fluidic channel needs to be effectively sealed, ideally with the same material or with similar wettability.
Figure 2 – Automated fixture to singulate and insert beads into the well
Manufacturing solutions for sealing channels include pressure-sensitive adhesives, gluing with UV curing, selective laser soldering, or multi-shot molding. The choice of sealing method may require 100% testing of the sealing function during production.
Ensuring heating efficiency and uniformity is essential when the cartridge is inserted into the reader. The heat spreader, typically made of aluminum or copper, is responsible for raising and lowering the temperature of the reaction well. The mass of the heat spreader should be minimized to reduce thermal load and energy consumption while maintaining even temperature distribution.
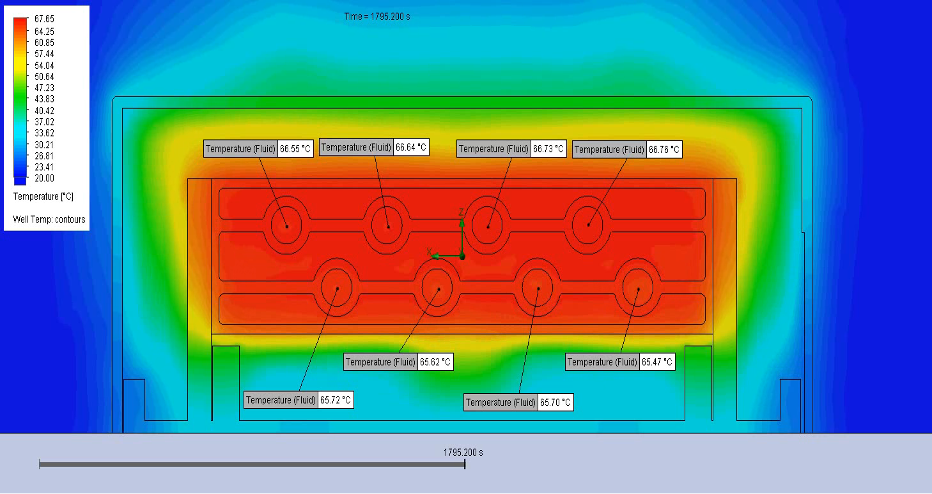
Thermal analysis and simulation can aid in determining the optimal thickness and material properties of the heat spreader.
Figure 3 – Thermal simulation of a cartridge with eight wells
Designing the heat spreader with the optimal thickness ensures even temperature distribution and uniform heating. Proper thermal analysis and simulation contribute to the overall performance of the cartridge-reader system. Integrating fans can expedite the cooling process, but noise levels and regular maintenance of air filters need to be managed to ensure a clean and efficient cooling system.
Example of how material thickness impacts heating time
Heat Spreader Thickness | 4mm | 2mm | 1mm | 0.5mm | 0.25mm |
Time to stable temperature | 164s | 107s | 79s | 65s | 57s |
Transduction
In the transduction step, the design of the cassette material must be transparent on one side for optical measurements while also providing effective heating through an opaque metallic heat spreader. Resolving these conflicting requirements demands innovative engineering solutions.
To strike a balance between thermal efficiency and optical accessibility, the material selection and design of the metallic heat spreader need to be carefully optimized.
One potential approach is to incorporate features like windows or transparent sections in the cassette material to allow light to pass through while still providing effective heating through the heat spreader. Accurate optical simulations can also allow for the use of the other side of the well for optical measurements.
An alternate approach is the adoption of a printed flexible heater directly attached to the cartridge. This eliminates the thermal interface to the reader and replaces it with an electrical interface to power the heating resistor via spring contacts. The printed flexible heater reduces thermal mass and resistance, enabling more precise and responsive temperature control. It ensures quick and accurate temperature control during thermal cycling.
Heating also plays a significant role in consuming energy in the system, and all of it is ultimately wasted. This has implications for the selection of battery capacity in portable devices, influencing the size and weight of the solution. It also contributes to a higher carbon footprint.
To address energy consumption and carbon footprint concerns, it is important to optimize the heating system for efficiency. This can involve using innovative heating
technologies, such as printed flexible heaters, that provide finer temperature control and reduce energy waste. Additionally, exploring alternative power sources or energy-saving strategies can help minimize the overall energy consumption of the system.
Measurement
When converting the emitted light intensity into a numerical value, the optical system design is crucial, requiring proper collection and focusing of laser light or LEDs onto the liquid sample. The wavelength of the light source must align with the acceptance bandwidth of the labeled reagent.
Additionally, point-of-care diagnostic cartridges may have multiple wells for testing against different molecules, requiring careful design considerations such as including an empty chamber for self-testing and alignment checks.
To ensure accurate measurements, only one well at a time should be exposed to the light to avoid interference from other wells. Protecting the optics from ambient light is critical, and a chopping algorithm can be used to remove the baseline signal generated by stray light. Using a black enclosure will block any interfering light.
The excitation wavelength and emission spectrum often lie close together, requiring the prevention of direct line of sight between the LED light and the collecting photodiode.
Expensive optical filters are typically used to cut off the LED light.
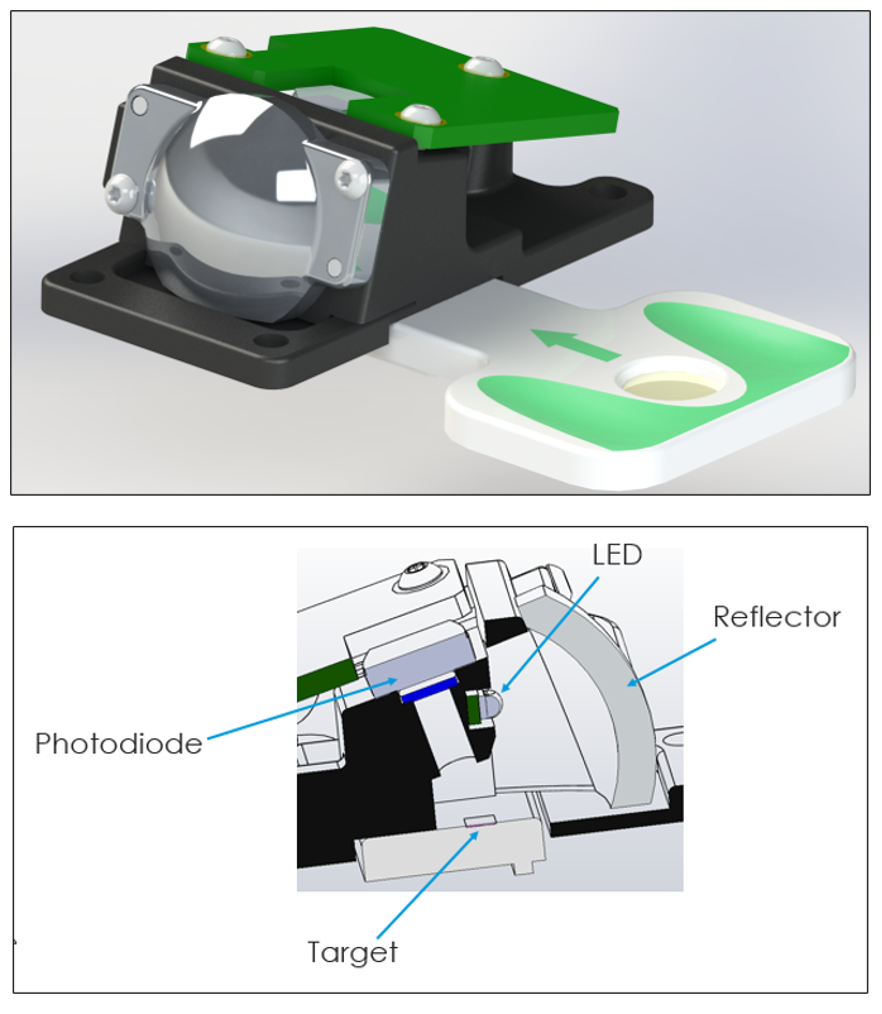
Figure 5 – 3D rendering and cross section of optical engine with inserted cartridge
During the amplification cycle, the electrical signal initially remains constant, and then a ramp emerges as the amplification reaches the appropriate level. Different amplification methods, such as PCR-based or RT-LAMP, generate different response patterns.
Optical design requires a system architecture approach, considering mechanical, electrical, and software aspects. Simulations and gage R&R analysis can help optimize the system. Multiplexing techniques can be employed to reduce the cost of optical-to-electrical conversion, either through time-sharing activation of light sources or utilizing multiple reagents emitting light at different wavelengths.
After the emitted light is focused on the photodiode, it is converted into an electrical signal that needs to be amplified and protected from external noise. Good electrical design, layout, and shielding are essential to prevent electromagnetic interference. The amplified signal is then converted into a numerical form by an analog-to-digital converter (ADC) for further software processing. The choice of ADC and its settings should be carefully considered for accuracy and precision.
Signal processing algorithms vary depending on the specific solution, ranging from simple linear calibration curves to more complex architectures based on machine learning. The goal is to ensure efficiency and cost-effectiveness while delivering reliable results. Finally, the ability to correlate results with those from a central lab is crucial in certain scenarios, allowing for comparison and analysis of temporal changes in measurements.
Display
For simple devices, green and red indicators are used to inform the user about the presence or absence of the analyte. In more complex devices, a color touch screen is employed to guide users through preparation steps, display machine status, and show quantitative measurements.
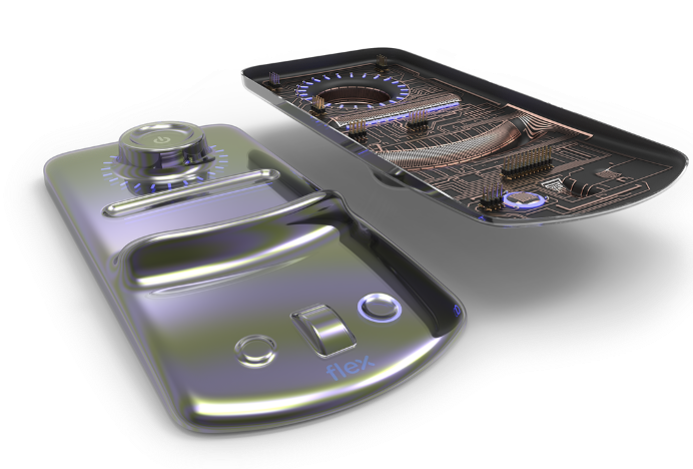
Touch interfaces for buttons are preferred as they are easy to clean and sterilize.
Touch and proximity sensing electronics are suitable for plastic enclosures, allowing for seamless integration of touch controls.
Figure 6 – Touch interface with conducive traces inserted as a film during molding process cartridge
Advancements in processing algorithms enable the recreation of rotary knobs and buttons on touch screens, providing a tactile experience with haptic feedback. A polycarbonate lens protects the display area, ensuring liquid ingress and Electrostatic Discharge (ESD) protection. Alternatively, connectivity options like Bluetooth allow for seamless connection to tablets or smartphones, creating a comprehensive interface without significantly increasing the cost. This leverages the familiarity of mobile devices for a user-friendly and intuitive experience. In settings with multiple readers, a tablet can be used to monitor and control all readers simultaneously, simplifying management and streamlining the testing process for increased efficiency and productivity.
Transfer
Point-of-care devices can benefit from incorporating wired connectivity ports or WiFi/5G capabilities, enabling significant data automation. Utilizing pre-qualified modules allows for modular solutions and potential upgrades, enhancing the device’s versatility. Wired or wireless connectivity enables direct uploading of data to a cloud-based system, streamlining data management.
The inclusion of a barcode reader ensures accurate and error-free data collection by efficiently capturing user information from QR codes or registration forms. To protect sensitive information, encryption functions and certificate signing can be implemented, enhancing security and privacy. Software upgrades may be necessary to support new cartridges or enable specific analyses, ensuring the device remains up to date. Radio frequency simulations help maintain reliable connectivity range in portable handheld devices, ensuring that the presence of the hand does not impact the device’s antenna efficiency.
Our expertise in optimizing design for manufacturability enables features for automated assembly from the outset, and guides customers through the selection of key components, for a reliable ramp-up phase through a prequalified supply chain.
— Marco De Angeli, Senior Director, Design, Process, and Technology Engineering, Flex
Other advancements in point-of-care technology
There are several exciting technologies under development that hold the potential to revolutionize the design and production of point of care diagnostic devices soon:
CRISPR technology
Boosting the creation of very accurate detection methods based on CAS enzymes, CRISPR opens the door to a fast process to build very custom and specific targets to be detected.
3D fast prototyping technology
Poised to enter high-volume manufacturing as an alternate process, this advancement offers the capability to create fluidic channels with faster speeds and keep the required resolution, ensuring the right surface quality and wettability. This can lead to more efficient and reliable diagnostic devices.
Quantum dots
A promising avenue for enhancing reagents and their fluorescent taggants. By leveraging quantum dots, the signal can be significantly boosted, allowing for better control of emitted light and simplifying the measurement process.
Additionally, quantum dots can enable the emitted spectrum to be engineered further apart from the excitation spectrum, reducing the need for costly optical filters. This will also enable multiplexing through frequency division, streamlining the overall optical architecture and component count.
RFID/NFC
RFID/NFC silicon technology is continuously evolving, becoming more feature-rich and integrated into microcontrollers. As the temperatures required for running the chemistry in diagnostic devices decrease, it opens up the possibility of fully disposable cartridges. In this scenario, energy harvesting from smartphones could power the entire measurement process, making point of care diagnostics even more accessible and convenient.
Environmentally friendly resins
Medical-quality resins are readily available in the market. These resins can be utilized in the next generation of disposable cartridges, reducing the overall CO2 footprint and contributing to more sustainable healthcare practices. Records, merging it with data collected at home using personal diagnostic devices and wearables. This powerful combination can lead to new insights in predictive and preventive medicine, enabling personalized healthcare tailored to each patient’s lifestyle and diagnostic data.
Cloud-integrated AI
The integration of AI at the cloud level allows for the analysis of data from personal Electronic Health Records, merging it with data collected at home using personal diagnostic devices and wearables. This powerful combination can lead to new insights in predictive and preventive medicine, enabling personalized healthcare tailored to each patient’s lifestyle and diagnostic data.
Conclusion
Each step and technology block within a point-of-care diagnostic device presents its own unique design challenges for engineering teams, but in addressing these challenges, medical device manufacturers can ensure accurate, reliable, and user-friendly results that meet the urgent demand for rapid and accurate testing.
By involving an experienced manufacturing and supply chain partner like Flex early in the product development cycle, manufacturers can move with greater agility and further enhance the design process.